Abstract
Members of the phylum Planctomycetota possess a plethora of intriguing and hitherto underexplored features including an enlarged periplasmic space, asymmetric cell division (“budding”), and a mostly undiscovered small molecule portfolio. Due to the large phylogenetic distance to frequently used and easily genetically accessible model bacteria, most of the established genetic tools are not readily applicable for the here-investigated bacterial phylum. However, techniques for targeted gene inactivation and the introduction of heterologous genes are crucial to investigate the cell biology in the phylum in greater detail. In this study, the targeted genomic modification of model planctomycetes was achieved by enforcing two types of homologous recombination events: simultaneous double homologous recombination for the deletion of coding regions and insertion-duplication mutagenesis for the introduction of foreign DNA into the chromosome. Upon testing the expression of commonly used fluorescent protein-encoding genes, many of the tested native promoters could not be harnessed for variation of the expression strength. Since also four commonly used inducible gene expression systems did not work in the tested model strain Planctopirus limnophila, a native rhamnose-dependent transcriptional regulator/promoter pair was established as an inducible expression system. The expanded molecular toolbox will allow the future characterization of genome-encoded features in the understudied phylum.
Key points
• Two recombination methods were used for the genetic modification of planctomycetes
• Commonly used fluorescent proteins are functional in model planctomycetes
• A rhamnose-dependent regulator was turned into an inducible expression system
Similar content being viewed by others
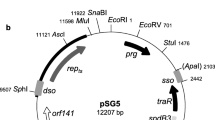
Explore related subjects
Discover the latest articles and news from researchers in related subjects, suggested using machine learning.Avoid common mistakes on your manuscript.
Introduction
Bacteria of the phylum Planctomycetota are increasingly recognized for their intriguing cell biology and potential biotechnological application. Despite being the fourth most prevalent bacterial phylum in soil (Delgado-Baquerizo et al. 2018), previous research has primarily focused on Planctomycetota in aquatic environments. In such ecosystems, members of the phylum commonly associate with various marine phototrophs (Bengtsson et al. 2012; Bondoso et al. 2014; Lage and Bondoso 2014; Vollmers et al. 2017), where they form dominant biofilms (Bengtsson and Øvreås 2010; Kohn et al. 2020) and metabolize polymeric carbon substrates (Jeske et al. 2013; Lachnit et al. 2013). Their prevalence on nutrient-rich surfaces in otherwise oligotrophic ecosystems, as for example oceans, is intriguing given their relatively slow growth compared to microbial competitors, e.g., members of the phylum Pseudomonadota (Frank et al. 2014; Wiegand et al. 2018).
In a joint effort, our team and others have isolated and described more than 100 novel strains from diverse environments in the last decade that have been added to the current open collection of ca. 130 strains (Devos et al. 2020; Kallscheuer et al. 2024; Wiegand et al. 2020). Previous investigations of their cell morphology suggested exceptions to existing models of diderm bacteria (Boedeker et al. 2017; Jeske et al. 2015). For example, they possess distinctive crateriform structures potentially involved in polysaccharide uptake (Boedeker et al. 2017). An enlarged periplasmic space might facilitate the subsequent digestion of internalized polysaccharides. Asymmetric cell division independent of the hallmark protein FtsZ is another distinctive feature of most members of the phylum (Jogler et al. 2012; Rivas-Marin et al. 2020; Wiegand et al. 2020). While planctomycetal cell walls contain peptidoglycan (Jeske et al. 2015; van Teeseling et al. 2015), genes involved in its biosynthesis are only partially present in planctomycetal genomes (Mahajan et al. 2020; Wiegand et al. 2020). In the limnic species Planctopirus limnophila, the peptidoglycan biosynthesis genes have even been shown to be non-essential (Rivas-Marin et al. 2023).
Bioprospection studies published in the last 5 years have started to uncover the untapped small molecule portfolio of the phylum (Graça et al. 2016; Jeske et al. 2016), which includes molecules with potential health-promoting bioactivities (Belova et al. 2020; Calisto et al. 2019; Kallscheuer and Jogler 2021). The ability to produce bioactive compounds, their large genomes, and the high number of genes of unknown function (Kallscheuer and Jogler 2021) make phylum members promising candidates for drug discovery and development. Recent discoveries include stieleriacines, potential biosurfactants (Kallscheuer et al. 2020; Sandargo et al. 2020), carotenoid pigments (Kallscheuer et al. 2019; Santana-Molina et al. 2022), an aromatic plant toxin (Panter et al. 2019), and alkylresorcinols of yet unknown function (Milke et al. 2024).
These recent discoveries in cell biology and small molecule biochemistry mark a transition phase from the descriptive characterization of novel strains to understanding fundamental molecular machineries and exploring potential biotechnological applications. The predicted ability of characterized strains to degrade complex polysaccharides suggests additional applications in industrial processes, such as biomass conversion and bioremediation (Boedeker et al. 2017; Klimek et al. 2024).
So far, genetic tool development for Planctomycetota has lagged behind other bacterial phyla. Main obstacles were the limited availability of axenic cultures, the requirement of complex media compositions, the natural low growth rate (typical generation times between 8 and 100 h), the aggregation behavior, and the natural resistance to common antibiotics (Godinho et al. 2019; Ivanova et al. 2021; Kallscheuer et al. 2021). Genetic tools are available for four species belonging to three different orders within the phylum Planctomycetota (Fig. S1) (Jogler et al. 2011; Rivas-Marín et al. 2016). Genetic manipulation was mainly based on random transposon-mediated insertion mutagenesis (Rivas-Marin et al. 2023) and the introduction of reporter genes (Rivas-Marín et al. 2016; Boedeker et al. 2017) as well as modification at single genomic loci (Erbilgin et al. 2014; Wiegand et al. 2020). To accelerate the research progress in this phylum, more robust genetic tools allowing the target-specific genetic manipulation of planctomycetes are urgently needed, and therefore must be established and further optimized for these bacteria.
In this study, an improved molecular toolbox is presented that is based on two homologous recombination strategies (Fig. 1) applicable for gene inactivation and introduction of foreign genes at defined loci. The combination of parts of a native regulatory circuit, fluorescent reporter genes, and selection markers was exploited for the construction of genetically modified strains of the limnic species P. limnophila and the marine species Stieleria maiorica.
Homologous recombination strategies used for gene inactivation and introduction of foreign genes. A Simultaneous double homologous recombination based on two recombination events between an up- and downstream region of a genomic target region (grey boxes). B Insertion-duplication mutagenesis based on a single homology region used to insert entire plasmid cassettes into the chromosome. The cat gene (encoding chloramphenicol acetyltransferase) as resistance marker is shown exemplarily. GOI, gene of interest; ori, origin of replication
Materials and methods
Medium preparation and cultivation
Escherichia coli DH5α was used for plasmid constructions and was cultivated in LB medium (5 g/L yeast extract, 10 g/L tryptone, 5 g/L NaCl) at 37 °C. The limnic model planctomycete P. limnophila DSM 3776 T was cultivated in limnic M3 medium (synonym: M3H NAG AFW), while the marine strain S. maiorica Mal15T was cultivated in marine M3 medium (synonym: M3H NAG ASW) (Wiegand et al. 2020). If required, kanamycin (50 mg/L), chloramphenicol (34 mg/L), or spectinomycin (100 mg/L) were added to the media. Solidified media were prepared by the addition of 15 g/L agar. For the planctomycete media, the agar was washed three times with ddH2O, autoclaved separately (121 °C, 20 min), and added after cooling down to < 60 °C. Bacterial growth was followed by measuring the optical density at 600 nm (OD600). For cultivation experiments with wild-type and genetically engineered strains of P. limnophila and S. maiorica, strains were first streaked on limnic and marine M3 agar plates, respectively, with the appropriate antibiotic (when applicable). Material from the plates was used for the inoculation of a preculture. All main cultures were inoculated from precultures to the indicated OD600.
DNA amplification and construction of plasmids
All bacterial strains and plasmids used in this study as well as their relevant characteristics are listed in Table S1. Oligonucleotides used in this study are listed in Table S2. Standard protocols of molecular cloning, such as PCR, DNA restriction, and ligation (Sambrook and Russell 2001), were carried out for recombinant DNA work. The amplification of DNA fragments for subsequent cloning into plasmids was performed using the Q5 polymerase Master Mix (New England Biolabs) based on the manufacturer’s protocol. Oligonucleotides were obtained from Metabion. The PCR clean-up was conducted using the NucleoSpin Gel and PCR Clean-up Mini Kit (Macherey–Nagel). Colony material of P. limnophila or S. maiorica or plasmids harboring the desired genes were used as template. FastDigest enzymes used for restriction and alkaline phosphatase (FastAP) for vector dephosphorylation were obtained from Thermo Scientific. The Rapid DNA Ligation Kit (Thermo Scientific) was used for ligation reactions and the constructed plasmids were isolated using the GeneJET Plasmid Mini-Prep kit (Thermo Scientific). Check-PCR was performed with the DreamTaq Green PCR Master Mix (Thermo Scientific) with the following program: initial denaturation 5 min, 95 °C; 33 cycles of denaturation (95 °C, 20 s), annealing (58 °C, 15 s) and elongation (72 °C, 1 min/kb), and a final elongation step (95 °C, 5 min). All constructed plasmids were verified by check-PCR using primers that bind outside of the inserted sequences and by DNA sequencing at Macrogen Europe.
Preparation of electrocompetent cells and transformation
Electrocompetent cells of P. limnophila were prepared from 50 mL of an exponentially growing culture (OD600 of 0.5–0.7) in limnic M3 medium. For S. maiorica Mal15T, 200 mL of an exponentially growing culture (OD600 of 0.5–0.7) in marine M3 medium was harvested. Cells were harvested by centrifugation (4600 × g, 4 °C, 20 min) and washed twice with sterile 10% (v/v) glycerol. Cells obtained after the second washing step were mixed with (linearized) plasmid DNA and transferred to a Gene Pulser Electroporation Cuvette with 0.2 cm gap (Biorad). The electroporation was performed with a GenePulser Xcell (Biorad) with the following parameters: 2500 V, 25 µF, 200 Ω, pulsing time 5 ms. The cell suspension was immediately transferred to 4 mL limnic M3 medium (P. limnophila) or marine M3 medium (S. maiorica) and cultivated for 3 h at 28 °C on a rotary shaker. The cells were harvested by centrifugation (4600 × g, 20 °C, 6 min) and ultimately streaked on plates containing the appropriate antibiotics that were subsequently incubated at 28 °C. Colonies obtained after 7–10 days were re-streaked on antibiotic-containing agar plates and checked for the presence of the expected modification in the genome using check-PCR.
Gene inactivation by two simultaneous crossing-over events
For the targeted gene inactivation by two simultaneous crossing-over events, the flanking regions up- and downstream of the coding sequence of a specific gene (approximately 1500 bp each) were amplified and subsequently cloned into the plasmid pCJ003x(kan), pCJ003x(cat), or pCJ003x(spec), depending on the chosen selection marker. The amplified homology regions still included the first and last 24 nucleotides of the coding sequence of the inactivation target. In the final plasmid, the inserted homology arms flank the resistance gene including its promoter. Prior to the transformation of electrocompetent cells, 2 µg of plasmid DNA was linearized with a single-cutting restriction enzyme that cleaves outside of the assembled insert region (upstream homology region–resistance gene–downstream homology region) and was purified with the NucleoSpin Gel and PCR Clean‑up Kit (Macherey–Nagel).
Introduction of heterologous genes by insertion-duplication mutagenesis
For testing the expression of heterologous genes, entire plasmid cassettes were inserted into the genome. This was achieved using an insertion-duplication strategy based on a single crossing-over event. For this purpose, a ca. 1300-bp fragment amplified from the genome of P. limnophila was inserted into the plasmid pASK-IBA3C (IBA Lifesciences) that was previously modified by removal of the anhydrotetracycline-inducible tetA promoter. The resulting plasmid pTH13mod was used in subsequent cloning steps for the insertion of constitutive and inducible promoters in combination with fluorescent reporter genes. Preparation of competent cells and transformation of P. limnophila were performed in the same manner as described above, but 2 µg of circular plasmid DNA was used for the transformation.
Isolation of genomic DNA, Illumina sequencing, and read mapping
DNA extraction and quality control were performed according to a previously published workflow (Wurzbacher et al. 2024). Illumina NovaSeq sequencing of P. limnophila DSM 3776 T wild type and mutant strains was performed by Eurofins Genomics (Luxemburg). The obtained reads (per strain approx. 5 million read pairs, 2 × 150 bp) were uploaded to the Galaxy Europe web server (The Galaxy Community 2022). All reads passed the quality check with FastQC version 0.11.9 (https://github.com/s-andrews/FastQC) and were further processed without additional trimming and filtering.
The reads of P. limnophila DSM 3776 T were used to polish the NCBI reference genome (accession number: ASM9210v1). Read mapping to the reference was done using BWA-MEM2 version 2.2.1 (Li and Durbin 2009, 2010) with the “Simple Illumina Mode”. Genome polishing was performed using Pilon version 1.20 (Walker et al. 2014). The polished reference genome of the P. limnophila was annotated with prokka version 1.14.6 (Seemann 2014). To analyze the gene inactivation mutants, the sequencing reads of the mutant strains were mapped to the polished reference genome of P. limnophila using BWA-MEM2 version 2.2.1 (Li and Durbin 2009, 2010) with the “Simple Illumina Mode”. The results were visualized with the IGV genome browser (Robinson et al. 2017) and manually analyzed.
Fluorescence microscopy setup and image analysis
Cell mounting, microscopy, post-processing, and image analysis were performed as described previously (Haufschild et al. 2024) with minor modifications. Briefly, 2 µL of the cultures were placed on 1% (w/v) agarose cushions on object slides and covered with a coverslip. Cells were imaged with a Ti2 inverse microscope (Nikon) equipped with a Nikon N Plan Apo λ 100x/1.45 Oil objective, a Hamamatsu Orca-flash 4.0 LT Plus camera, and a Lumencor light source. Images were split in FIJI (Schindelin et al. 2012) and analyzed in BacStalk (Hartmann et al. 2020) with 0.065 μm as pixel size, 25 pixels as cell size, and 15 pixels as minimal cell size for cell segmentation and mean fluorescence intensities per cell were extracted. The obtained data was visualized with SuperPlotsOfData (Goedhart 2021).
Fluorescence microscopy of fluorescent reporters
For microscopy of cells of P. limnophila and S. maiorica harboring a chromosomally integrated fluorescent reporter, the following combination of fluorescent reporters, LED wavelengths, and filter cubes were used: msfTurquoise2ox (440 nm, Semrock CFP-2432C); mNeonGreen, GFPmut2, and msfGFP (470 nm, Semrock FITC-3540C), mVenus (508 nm, Semrock YFP-2427B), mCherry (555 nm, mCherry-C), and HaloTag with OregonGreen Ligand (470 nm, Semrock FITC-3540C). For staining and washing of P. limnophila cells expressing the HaloTag-encoding gene, the manufacturer’s recommendations were followed. Images were transferred to FIJI where intensity and brightness were adjusted and scale bars were added.
Analysis of gene expression strength of native promoters from P. limnophila
Cells of P. limnophila strains constructed for the gene expression analysis were cultivated for 2 days in precultures and main cultures were inoculated to an initial OD600 of 0.1. After 1 day of incubation, the OD600 was measured; 500 µL of cells was centrifuged for 3 min at 17,000 g, washed with sterile distilled water; and fluorescence intensity of 150 µL culture was measured with an Infinite MPlex 200 plate reader (Tecan). Prior to measurement, the culture was homogenized by 5-s orbital shaking followed by 8-s linear shaking. Measurement settings were as follows: 499-nm excitation wavelength (9-nm bandwidth), 530-nm emission wavelength (20-nm bandwidth), gain value of 14, 15 flashes, and 20-µs integration time. Obtained fluorescence signals were corrected for the autofluorescence of the medium and normalized to the OD600 before mean values were calculated. Negative values for fluorescence intensities obtained after autofluorescence subtraction were regarded as a fluorescence intensity of zero. The data was further normalized to the fluorescence intensity of the Pgap construct (= 100%). The fluorescence intensity values measured with the MPlex platereader were visualized with RStudio (RStudio 2022) employing the ggplot2 package (Wickham 2016). For fluorescence microscopy, LED and filter settings were used as described above for mNeonGreen, the exposure time was set to 400 ms for all samples. The mean fluorescence intensity of cells (in total 300 cells per condition) was analyzed as described above.
Analysis of the rhamnose-inducible gene expression system in P. limnophila
P. limnophila strains with either the empty vector control or the plasmid bearing the rhamnose-inducible construct PpvmA-pvmA-PpvmB-msfgfp introduced into the chromosome were inoculated in medium containing glucose and N-acetyl glucosamine (NAG). After 2 days of growth, cells were incubated to an OD600 of 0.05 in limnic M3 medium with glucose, but without NAG. Two days later, cells were inoculated to an OD600 of 0.1 (main culture) in limnic M3 medium containing the respective conditions comprising either 0.2% (w/v) rhamnose, 0.1% (w/v) rhamnose and 0.1% (w/v) glucose, 0.2% (w/v) glucose, or none of the two sugars. After 24 h, cells were imaged with the setup described above using the LED/filter cube setting for msfGFP; exposure time was the same for all samples. For image processing, fluorescence brightness and contrast settings of all images were adjusted manually to the same values and scale bars were added using FIJI. Image analysis was conducted as described above analyzing 400 cells per condition in total (200 cells of two biological replicates). The same experimental and analysis design was used for the rhamnose concentration-dependence experiment. The only difference were the sugar concentrations (w/v) added to the medium, either 0.2% glucose, 0.05%, 0.1%, 0.2%, 0.5%, or 1% rhamnose. In total, four replicates with 150 cells per replicate were analyzed.
Results
Construction of single gene deletion mutants in limnic and marine model planctomycetes
The easiest way to investigate the role of non-essential protein-coding genes is the construction of respective gene inactivation mutants by interrupting the open reading frame or removing it (Fig. S2). Depending on the inactivation target, the deletion can result in a detectable phenotype, e.g., the inability to degrade a certain carbon source or to form a characteristic pigment. The presqualene diphosphate synthase HpnD catalyzes the initial step during synthesis of the triterpene squalene from farnesyl pyrophosphate. Squalene serves as precursor for C30 carotenoid biosynthesis in P. limnophila (Santana-Molina et al. 2022). Hence, deletion of the encoding gene should abolish carotenoid formation and yield white colonies. The deletion was tested in the type strains of the pink-pigmented and limnic model species P. limnophila (hpnD locus tag Plim_2244, UniProt entry D5SNF6) and the salmon-colored marine species S. maiorica (hpnD locus tag Mal15_66750, UniProt entry A0A5B9MNP2). A kanamycin resistance gene was used for the selection of recombinant clones of P. limnophila while inactivation in S. maiorica required a chloramphenicol resistance gene (cat) due to resistance of the species to aminoglycosides. One week after transformation of the two strains by electroporation, approximately 100 and 20 recombinant clones were obtained for P. limnophila and S. maiorica, respectively. All obtained colonies lacked the pink or salmon pigmentation of the respective wild-type strains indicating that the recombination event occurred at the correct genomic location in all obtained colonies. This was also confirmed by check PCR performed with three colonies per strain that were re-streaked to check for maintenance of the resistance marker. The linear DNA carrying the flanking homology regions of the inactivation targets successfully enforced simultaneous homologous recombination events (Fig. 1A) that ultimately led to the exchange of hpnD with the resistance marker gene (Fig. 2, S3). The recombination did not require any foreign recombinases.
Target-specific inactivation of genes. The presqualene diphosphate synthase HpnD catalyzes the initial step during C30 carotenoid biosynthesis in planctomycetes. The deletion of the encoding gene abolished carotenoid formation and yielded white colonies in P. limnophila and S. maiorica. The wild type of both species is shown for comparison
Modifications at up to three genomic loci can be combined in P. limnophila
The here-used gene inactivation strategy does not include a counterselection step to remove the resistance marker gene. Since such a marker is currently not available for the phylum, the resistance marker gene needs to remain in the genome of the recombinant strain. However, in case that a strain is susceptible to other commonly used antibiotics with established resistance markers, it should be possible to combine modifications at different loci in one strain. Unfortunately, S. maiorica turned out to be naturally resistant to kanamycin, spectinomycin, tetracycline, and β-lactam antibiotics (such as ampicillin and carbenicillin) and was not considered for testing the introduction of combined genetic modifications at two or more loci. In contrast, the limnic P. limnophila is susceptible to kanamycin, spectinomycin, and chloramphenicol and only resistant to β-lactam antibiotics.
After an inspection of the P. limnophila genome for inactivation targets that have similar putative functions but are not essential under laboratory-scale cultivation conditions, genes encoding PilQ-like proteins turned out to be suitable candidates. PilQ proteins are involved in the formation of type IV pili (T4P) by assembly into homo-multimeric structures constituting the outer membrane pore of the T4P apparatus (Berry et al. 2012). From constructed single gene deletions, it was clear that the three putative pilQ genes Plim_0794, Plim_3284, and Plim_3688 are not essential under standard cultivation conditions and that the tested resistance markers for chloramphenicol and spectinomycin are functional in P. limnophila. The expected sequence of these strains was first confirmed by check-PCRs and later via genome sequencing and read mapping to the modified loci. Off-target effects could thereby be excluded as well (Fig. S4A). By the subsequent deletion of the three genes, the triple deletion mutant P. limnophila ΔPlim_3688::kanR ΔPlim_0794::cat ΔPlim_3281::specR could be obtained. The expected genotype of the strain was confirmed by check PCR-based amplification of the modified loci (Fig. S4B). After having confirmed that deletion mutants can be obtained in the way described, the cloning plasmids pCJ003x(kan), pCJ003x(cat), and pCJ003x(spec) that were used for the insertion of the homology regions flanking the inactivation target were modified. Plasmid sequences not required for replication in E. coli were removed and an additional terminator sequence downstream of the resistance gene was introduced along with improved multiple cloning sites for the insertion of the upstream and downstream homology regions. The stream-lined plasmids pDEL(kan), pDEL(cat), and pDEL(spec) (Fig. S5) will be used for the future construction of deletion mutants in planctomycetes.
Commonly used fluorescent reporters are functional in planctomycetes
In previous studies, green fluorescent protein (GFP)-encoding genes have been introduced into four planctomycetotal species (Boedeker et al. 2017; Jogler et al 2011, Rivas-Marín et al. 2016). Although this led to a constitutive expression of the GFP-encoding gene as a weak dimer in the cytoplasm, the strategy is not well-suited for detailed protein function, localization, or interaction studies. Instead, studies employing wide-field and super-resolution microscopy methods based on monomeric fluorescent proteins are essential to address biological questions and require targeted modifications in the genome. For this reason, an insertion-duplication mutagenesis strategy was applied for the introduction of entire plasmids into the genome of P. limnophila using site-specific single homologous recombination (Fig. 1B). The starting point for these experiments was the empty vector pASK-IBA3C, a plasmid originally constructed for an inducible gene expression in E. coli. Since the hpnD gene locus Plim_2244 is accessible for genetic modifications, a 1300-bp sequence covering the upstream flanking region and the first 150 bp of the hpnD open reading frame was cloned into the plasmid outside of its multiple cloning site. The inserted DNA from P. limnophila functions as a “landing site” for the introduction of the entire plasmid into the genome. The anhydrotetracycline-inducible promoter from the original plasmid was removed to yield pTH13mod (Fig. 3A). To test the insertion into the chromosome of P. limnophila, the native constitutive promoter of the glyceraldehyde-3-phosphate dehydrogenase gene (Pgap) and the previously tested gfpmut2 were cloned into the plasmid yielding pTH13mod_Pgap-gfpmut2. The chosen promoter-reporter combination was already shown to be functional in a previous study (Boedeker et al. 2017). After transformation of electrocompetent P. limnophila cells, a low number of colonies (< 10) per plate was obtained. Microscopic analyses confirmed the expression of the gene gfpmut2 in the three tested clones of the recombinant strain. The fluorescence signal occurred in the cytoplasm whereas invaginations of the periplasm were not stained by the cytoplasmic GFPmut2 (Fig. 3B). To expand the set of functional fluorescent proteins, a range of different commonly used proteins was tested (Table 1). Candidates were chosen based on various criteria including brightness, multimerization, activity in the cytoplasm and/or periplasm, and suitability as partner for translational fusions or for the use with exchangeable ligands (enabling pulse-chase labelling or the use of high-resolution fluorescence microscopy ligands). The selected set included genes encoding msfTurquoise2ox (msfTq2ox), mNeonGreen, mVenus, mCherry, msfGFP, and the multi-purpose HaloTag that were individually inserted into the genome of P. limnophila. Like GFPmut2, all other tested reporter proteins folded and matured properly as confirmed by fluorescence signals in the cytoplasm (Fig. 3B).
Duplication-insertion mutagenesis in P. limnophila results in the expression of various fluorescent protein-encoding genes. A The expression vector pASK-IBA3C was used for the construction of pTH13mod by removal of PtetA and the f1-ori and replacement for an improved multiple cloning site and a region with homology to the hpnD locus of P. limnophila (purple). Unique restriction sites used for cloning are shown in the plasmid maps. B Epi-fluorescence images of P. limnophila cells show the expression of various reporter genes. S. maiorica with a stably integrated gfpmut2 gene was constructed with a modified protocol of the gene deletion strategy via two homologous recombination events (see text for details). OG, Oregon Green
Construction of a Stieleria maiorica strain with constitutive expression of gfpmut2
The handling of S. maiorica in the lab is quite challenging. The type strain Mal15T forms large amounts of extracellular polymeric substance (EPS) which renders it difficult to harvest cells by centrifugation. However, a minimum of two washing steps is crucial to remove salts from the marine medium prior to the electroporation. From 200 mL culture broth, only a tiny cell pellet remained after the two washing steps and most cells embedded in the EPS were lost during removal of the supernatant. Although the pTH13mod-based insertion-duplication mutagenesis may in principle also work for S. maiorica, the protocol was not followed for this strain. This is due to the following reasons: (i) The insertion of entire plasmid cassettes in P. limnophila worked at least one order of magnitude worse than the enforced double homologous recombination strategy and it was not possible to compensate this by the collection of sufficient cells of S. maiorica after centrifugation; (ii) plasmids introduced by insertion-duplication mutagenesis can spontaneously excise from the genome by reversal of the homologous recombination event and this can only be prevented by maintaining the selection pressure (supplementation of antibiotics). Since the gfpmut2-expressing strain is planned to be used for co-cultivation experiments with antibiotic-susceptible interaction partners, a modified version of the double homologous gene deletion strategy was used for strain construction. For this purpose, the gfpmut2 gene was used and the hpnD locus was targeted. The final plasmid used for S. maiorica strain construction contained the following insert: upstream homology arm of hpnD (ca. 1500 bp)–promoter–chloramphenicol resistance gene (cat)–Pgap (from P. limnophila)–gfpmut2–downstream homology arm of hpnD (ca. 1500 bp). The transformation with the plasmid linearized outside of the insert sequence yielded a single white S. maiorica colony that showed the ΔhpnD phenotype (lack of pigmentation, genotype confirmed by check PCR) and a fluorescence signal in the cytoplasm (Fig. 3B). The protocol is hence suitable for the stable introduction of foreign DNA into more challenging strains. By choosing different homology regions, foreign DNA can also be inserted into intergenic regions and does not necessarily need to be combined with the inactivation of a protein-coding gene.
Variation of the gene expression strength using alternative promoters in P. limnophila
After having established the protocol for the pTH13mod-based introduction of foreign DNA into the chromosome of P. limnophila and subsequent expression of fluorescent reporters, the next step included the testing of different native promoters aiming at different transcription rates of tested genes of interest. This becomes important for lowering the expression level of genes that code for proteins that show toxic effects to the host or interfere with essential cell biological processes, e.g., cell division. The glyceraldehyde-3-phosphate dehydrogenase promoter Pgap (also referred to as gapdh promoter) is frequently used in various genetically engineered bacteria because of its strong and constitutive expression during the exponential growth phase (Meyers et al. 2019; Olson et al. 2015; Pátek et al. 2003). Five different native P. limnophila promoters with assumingly lower natural transcription rates were chosen for the subsequent experiments in combination with mNeonGreen as reporter, namely PdapA (4-hydroxytetrahydrodipicolinate synthase gene dapA, lysine biosynthesis) PmurA (UDP-N-acetylglucosamine 1-carboxyvinyltransferase gene murA, peptidoglycan biosynthesis), PrecA (recA gene, involved in homologous recombination, DNA repair and competence), PfliA (RNA polymerase sigma factor gene controlling the expression of flagella-related genes), and Ptuf (gene encoding the elongation factor Tu, protein biosynthesis) (Fig. 4A). The assumed expression mode of the genes is constitutive (dapA, murA, tuf), cell-cycle regulated (fliA, since the flagellum is only formed once the daughter cell is released from the mother cell), or stress-regulated (recA). To estimate the strength of the chosen promoters, the −35 and −10 regions predicted by bprom (Softberry Inc.) were compared against the consensus sequences of σ70-dependent promoters (Fig. 4A). Based on this analysis, Pgap showed the highest similarity to the consensus sequence (two mismatches) while PfliA, PmurA, and Ptuf showed the lowest similarity (five mismatches each).
Testing of native promoters for alternation of the reporter gene expression levels in P. limnophila. A Predicted promoter sequences and comparison against the consensus sequence of σ70-dependent promoters. The predicted −35 and −10 regions of the promoters are shown in green. B Fluorescence intensity measurement on population level from cells harboring one of the selected promoter-reporter constructs. C Single-cell fluorescence intensity analysis of P. limnophila cells expressing the mNeonGreen reporter gene under the control of the selected promoters. Each circle represents one measured cell. ev, empty vector control; mNG, monomeric NeonGreen
Correct clones could be obtained for all chosen promoters except Ptuf, which did not give colonies of recombinant P. limnophila cells. To test the strength of the promoters in the obtained clones, fluorescence intensity was measured on a population level using a plate reader. As expected, the Pgap construct yielded the strongest fluorescence on population level; however, the fluorescence intensity of the other four promoters fell in the range of the autofluorescence of the empty vector control strain (Fig. 4B). The promoter strength could differ depending on the life cycle stage or stress level experienced by individual cells. Therefore, the fluorescence intensity was also checked on single-cell level employing wide-field fluorescence microscopy. The single-cell data showed a similar distribution of fluorescence levels, thereby confirming the results of the plate reader experiment (Fig. 4C).
Commonly used inducible expression system do not function in P. limnophila
Inducible expression systems have decisive advantages over constitutive promoters in terms of flexibility. The time point of induction and the expression rate can be controlled by the externally supplemented inducer. The proper activity of an inducible expression system typically requires the synthesis of a functional transcriptional regulator protein, the efficient uptake of the inducing metabolite, and the recognition of the regulated promoter by the host’s transcription initiation complex. Plasmids harboring the following regulator/regulated promoter pairs were introduced into the genome of P. limnophila in combination with the msfTq2ox- and/or the mCherry-encoding gene: LacI/Ptac (inducer: isopropyl β-d−1-thiogalactopyranoside, IPTG) (Stansen et al. 2005), NagR/PnagAa (inducer: salicylic acid) (Kruse et al. 2024), XylS/Pm (inducer: salicylic acid) (Hogenkamp et al. 2022), and TetR/PtetA (inducer: anhydrotetracycline) (Skerra 1994). Although all plasmid-based systems were confirmed to be functional in E. coli, none of the systems worked in P. limnophila. Either no recombinant clones could be obtained after several rounds of transformation (both salicylate-inducible systems) or no expression of the reporter genes was detected even in presence of the maximal concentration of the inducers (LacI/Ptac and TetR/PtetA systems) (data not shown).
A native sugar-dependent regulator/promoter pair was turned into an inducible gene expression system
Since none of the commonly used inducible expression systems worked in P. limnophila, characterized regulatory circuits in this bacterium were evaluated as potential inducible gene expression systems. P. limnophila can use rhamnose and fucose as carbon and energy sources. The degradation of these monosaccharides has been shown to take place in a bacterial microcompartment (BMC) due to the toxicity of one of the pathway intermediates (Erbilgin et al. 2014). The genes constituting the rhamnose/fucose catabolic pathway are encoded along with BMC structural protein-encoding genes in the pvmABDEGHIJKLMNO cluster (Fig. 5A). The first gene of the cluster codes for the putative DeoR-family transcriptional regulator PvmA (Plim_1758, UniProt entry D5SXM1) that likely responds to rhamnose or one of the early pathway intermediates to induce the expression of the cluster. To turn the natural cluster into an inducible expression system, the predicted promoter regions and the open reading frame of pvmA were amplified and cloned into pTH13mod in a way that the start codon of the msfgfp gene is at the same position as that of pvmB in the original cluster (pvmB and subsequent genes were omitted) (Fig. 5B). The recombinant P. limnophila strain, now harboring PpvmA-pvmA-PpvmB-msfgfp in addition to the unmodified natural cluster, was cultivated in the presence of different concentrations and combinations of rhamnose and glucose as well as in the absence of an additional carbon source (Fig. 5B, C). Initial concentrations of 0.2% (w/v) rhamnose in the medium induced the expression of msfgfp and led to a strong fluorescence signal per cell that was homogeneously distributed throughout the cytoplasm (Fig. 5C, D) indicating a strong expression of the fluorescent reporter gene. When rhamnose was provided in addition to glucose, a lower expression level than for rhamnose alone was obtained; however, the expression was slightly higher than that in a culture without glucose or rhamnose. When only glucose was provided, the measured fluorescence was lower than that in a culture without glucose or rhamnose. This points towards the presence of additional catabolite repression mechanisms, suggesting a diauxic growth behavior of the strain. The presence of such a system appears physiologically reasonable since glucose degradation (glycolysis) does not require the additional expression of proteins forming a BMC and is therefore less costly. An empty vector control did not show any increase of the fluorescence signal over the autofluorescence level regardless of the amount of rhamnose or glucose present in the medium (Fig. 5D). To characterize the system in greater detail, the expression strength of the system was tested with different rhamnose concentrations ranging from 0.05 to 1% (Fig. 6). When 0.05%, 0.1%, or 0.2% rhamnose were supplied, the fluorescence intensity was similar (mean values of 6100 a.u., 5830 a. u., and 5970 a.u., respectively). Higher concentrations of rhamnose (0.5% and 1%) resulted in a decreased fluorescence intensity (mean values of 4990 a.u. and 4410 a.u., respectively), and the sole presence of 0.2% glucose showed a low fluorescence intensity (mean value of 1780 a.u.).
A native rhamnose-dependent promoter/regulator pair from P. limnophila displays differential expression of a fluorescent reporter when induced with rhamnose. A Genetic organization of the rhamnose catabolic cluster in P. limnophila including predicted promoters. B Organization of the constructed rhamnose promoter-reporter gene construct. The nucleotide sequence of the predicted −35 and −10 regions of the promoters are depicted in green. C Epi-fluorescence microscopy images showing altered expression of the reporter gene msfgfp upon induction of the promoter/regulator pair with rhamnose (Rh), glucose (Glc), a combination of rhamnose and glucose or without glucose or rhamnose. D Single-cell fluorescence intensity analysis of P. limnophila depicting the differential expression of msfgfp upon induction with rhamnose, glucose, the combination of rhamnose and glucose or without glucose or rhamnose. ev, empty vector control
The expression level of the native rhamnose-dependent promoter/regulator pair depends on the rhamnose concentration. Fluorescence intensity single-cell analysis of P. limnophila cells when incubated with varying concentrations of rhamnose (in w/v). In total, four replicates with 150 cells each were analyzed. Glc, glucose; Rh, rhamnose
Discussion
Planctomycetes are a group of bacteria that exhibit unique cellular and genomic features, making them intriguing but also challenging for genetic research. The current lack of robust genetic tools for planctomycetes can be attributed to several factors including their slow growth (generation times of at least 8 h), specialized growth requirements, limited knowledge of genetic mechanisms, inherent resistance to several classes of antibiotics, and a currently relatively small research community working with members of the phylum. Planctomycetes challenge many long-standing cell biological dogmas (Boedeker et al. 2017; Rivas-Marin et al. 2023) and their metabolism remains largely unexplored (Kallscheuer and Jogler 2021). To tackle open research questions, we here provide an improved molecular toolbox enabling the introduction of (multiple) genetic modifications and engineered (inducible) expression of (heterologous) genes.
The homologous recombination for deletion of genes by two simultaneous crossing-over events yielded more than 100 colonies in P. limnophila while the insertion-duplication mutagenesis approach for the insertion of entire plasmid cassettes worked one order of magnitude less efficient in our hands, typically yielding less than ten colonies per transformation reaction. The obtained differences are probably related to steric effects caused by the size of the plasmid compared to the shorter linearized fragment with up- and downstream homology regions. In addition, a competing excision reaction can occur after the single recombination event. This ultimately leads to the loss of the inserted plasmid sequence phenotypically visible in a lower number of obtained colonies. During the construction of deletion mutants, the number of obtained colonies typically decreased with the size of the inactivation target, i.e. the distance of the chosen up- and downstream homology arms. The crossing-over events appear to work best in cases in which the inactivation target is similar in length to the inserted resistance cassette. Larger inactivation targets might require secondary structure (hairpin) formation of the genomic DNA that could reduce the efficiency. For challenging strains, for which the less-efficient insertion-duplication mutagenesis fails, the gene deletion approach can also be exploited for the stable insertion of foreign DNA fragments (as shown for gfpmut2 in S. maiorica).
The current need for the introduction of heterologous genes into the genome is based on the lack of a replicative plasmid for model strains. Plasmids are commonly found in members of the order Isosphaerales in the phylum (some strains harbor up to five plasmids) and even the type strain of P. limnophila harbors an extrachromosomal element (prophage). However, the exact elements required for plasmid replication and distribution to the daughter cell only start to be unveiled (Quiñonero-Coronel et al. 2024). Like for the tested expression systems, replication machineries of commonly used plasmids for E. coli or with broader host range do not work in P. limnophila.
Instead of the established gene expression systems, a native rhamnose-responsive genetic circuit was turned into an inducible expression system based on the rhamnose/fucose catabolic pvm operon in P. limnophila. The gene cluster in this species was studied in greater detail (Erbilgin et al. 2014), but is also present in several other members of the phylum including S. maiorica. The genetic organization suggests that pvmA (encoding a DeoR-family transcriptional regulator) might be part of the operon. Such an organization has, e.g., been observed in the DeoR-family regulator-encoding gene rhaR in the rhamnose catabolic operon in Bacillus subtilis (Hirooka et al. 2015). An indication for a similar genomic setup in P. limnophila is the short intergenic sequence of 46 bp between pvmA and pvmB that is much smaller compared to intergenic regions of many of the other genes in the predicted operon. However, the pvmA–pvmB intergenic region harbors a predicted promoter with the −35 and −10 region similar to the respective consensus sequences for σ70-dependent promoters. Thus, instead of the 13-gene operon, the cluster might be expressed as two transcripts, one monocistronic mRNA harboring pvmA and the other one harboring pvmBDEGHIJKLMNO. The exact mode of action of PvmA cannot be answered from the genetic organization alone; however, most of the characterized DeoR-family regulators including RhaR from B. subtilis act as repressors (Hirooka et al. 2015). After having cloned the rhamnose-inducible construct with msfgfp as reporter, it turned out that the gene was strongly expressed in E. coli without the addition of rhamnose. This might reinforce PvmA being a transcriptional repressor with insufficient expression of its encoding gene in E. coli. In the case of a transcriptional activator, no strong expression in E. coli would have been expected in the absence of rhamnose or with a sufficient or insufficient expression of pvmA. Although functional in P. limnophila, the strong expression of the target gene in E. coli restricts the system to genes that are non-toxic for the used E. coli cloning strain.
With the tested set of seven fluorescent reporter proteins active in P. limnophila (some efficiently maturing in the periplasm), additional planctomycete-derived genetic circuits should first be characterized and can then be tested as inducible gene expression systems. In parallel, efforts to construct E. coli–P. limnophila shuttle vectors need to be intensified to facilitate the future investigation of cell biological principles in these non-model bacteria.
Data availability
Data and/or bacterial strains generated in this study are available upon reasonable request from the corresponding author. Nucleotide sequences of the used genes and promoters are provided in the Supporting Information.
References
Belova SE, Saltykova VA, Dedysh SN (2020) Antimicrobial activity of a novel freshwater planctomycete Lacipirellula parvula PX69T. Microbiology 89(5):503–509. https://doi.org/10.1134/S0026261720050045
Bengtsson MM, Øvreås L (2010) Planctomycetes dominate biofilms on surfaces of the kelp Laminaria hyperborea. BMC Microbiol 10:261. https://doi.org/10.1186/1471-2180-10-261
Bengtsson MM, Sjøtun K, Lanzén A, Øvreås L (2012) Bacterial diversity in relation to secondary production and succession on surfaces of the kelp Laminaria hyperborea. ISME J 6(12):2188–2198. https://doi.org/10.1038/ismej.2012.67
Berry J-L, Phelan MM, Collins RF, Adomavicius T, Tønjum T, Frye SA, Bird L, Owens R, Ford RC, Lian L-Y, Derrick JP (2012) Structure and assembly of a trans-periplasmic channel for type IV Pili in Neisseria meningitidis. PLoS Path 8(9):e1002923. https://doi.org/10.1371/journal.ppat.1002923
Boedeker C, Schuler M, Reintjes G, Jeske O, van Teeseling MC, Jogler M, Rast P, Borchert D, Devos DP, Kucklick M, Schaffer M, Kolter R, van Niftrik L, Engelmann S, Amann R, Rohde M, Engelhardt H, Jogler C (2017) Determining the bacterial cell biology of Planctomycetes. Nat Commun 8:14853. https://doi.org/10.1038/ncomms14853
Bondoso J, Balague V, Gasol JM, Lage OM (2014) Community composition of the Planctomycetes associated with different macroalgae. FEMS Microbiol Ecol 88(3):445–456. https://doi.org/10.1111/1574-6941.12258
Calisto R, Sæbø EF, Storesund JE, Øvreås L, Herfindal L, Lage OM (2019) Anticancer activity in planctomycetes. Front Mar Sci 5:499. https://doi.org/10.3389/fmars.2018.00499
Cormack BP, Valdivia RH, Falkow S (1996) FACS-optimized mutants of the green fluorescent protein (GFP). Gene 173(1):33–38. https://doi.org/10.1016/0378-1119(95)00685-0
Delgado-Baquerizo M, Oliverio AM, Brewer TE, Benavent-Gonzalez A, Eldridge DJ, Bardgett RD, Maestre FT, Singh BK, Fierer N (2018) A global atlas of the dominant bacteria found in soil. Science 359(6373):320–325. https://doi.org/10.1126/science.aap9516
Devos DP, Lage OM, Sutcliffe IC (2020) Bringing the diversity of Planctomycetes into the light: introduction to papers from the special issue on novel taxa of Planctomycetes. Antonie Van Leeuwenhoek 113(12):1715–1726. https://doi.org/10.1007/s10482-020-01499-y
Dinh T, Bernhardt Thomas G (2011) Using superfolder green fluorescent protein for periplasmic protein localization studies. J Bacteriol 193(18):4984–4987. https://doi.org/10.1128/jb.00315-11
Erbilgin O, McDonald Kent L, Kerfeld Cheryl A (2014) Characterization of a planctomycetal organelle: a novel bacterial microcompartment for the aerobic degradation of plant saccharides. Appl Environ Microbiol 80(7):2193–2205. https://doi.org/10.1128/AEM.03887-13
Frank O, Michael V, Pauker O, Boedeker C, Jogler C, Rohde M, Petersen J (2014) Plasmid curing and the loss of grip - the 65-kb replicon of Phaeobacter inhibens DSM 17395 is required for biofilm formation, motility and the colonization of marine algae. Syst Appl Microbiol 38(2):120–127. https://doi.org/10.1016/j.syapm.2014.12.001
Godinho O, Calisto R, Øvreås L, Quinteira S, Lage OM (2019) Antibiotic susceptibility of marine Planctomycetes. Antonie Van Leeuwenhoek 112(8):1273–1280. https://doi.org/10.1007/s10482-019-01259-7
Goedhart J (2021) SuperPlotsOfData-a web app for the transparent display and quantitative comparison of continuous data from different conditions. Mol Biol Cell 32(6):470–474. https://doi.org/10.1091/mbc.E20-09-0583
Goedhart J, von Stetten D, Noirclerc-Savoye M, Lelimousin M, Joosen L, Hink MA, van Weeren L, Gadella TWJ, Royant A (2012) Structure-guided evolution of cyan fluorescent proteins towards a quantum yield of 93%. Nat Commun 3(1):751. https://doi.org/10.1038/ncomms1738
Graça AP, Calisto R, Lage OM (2016) Planctomycetes as novel source of bioactive molecules. Front Microbiol 7:1241. https://doi.org/10.3389/fmicb.2016.01241
Hartmann R, van Teeseling MCF, Thanbichler M, Drescher K (2020) BacStalk: a comprehensive and interactive image analysis software tool for bacterial cell biology. Mol Microbiol 114(1):140–150. https://doi.org/10.1111/mmi.14501
Haufschild T, Kallscheuer N, Hammer J, Kohn T, Kabuu M, Jogler M, Wohlfarth N, Rohde M, van Teeseling MCF, Jogler C (2024) An untargeted cultivation approach revealed Pseudogemmatithrix spongiicola gen. nov., sp. nov., and sheds light on the gemmatimonadotal mode of cell division: binary fission. Sci Rep 14(1):16764. https://doi.org/10.1038/s41598-024-67408-9
Hirooka K, Kodoi Y, Satomura T, Fujita Y (2015) Regulation of the rhaEWRBMA operon involved in L-rhamnose catabolism through two transcriptional factors, RhaR and CcpA in Bacillus subtilis. J Bacteriol 198(5):830–45
Hogenkamp F, Hilgers F, Bitzenhofer NL, Ophoven V, Haase M, Bier C, Binder D, Jaeger KE, Drepper T, Pietruszka J (2022) Optochemical control of bacterial gene expression: novel photocaged compounds for different promoter systems. ChemBioChem 23(1):e202100467. https://doi.org/10.1002/cbic.202100467
Ivanova AA, Miroshnikov KK, Oshkin IY (2021) Exploring antibiotic susceptibility, resistome and mobilome structure of planctomycetes from Gemmataceae family. Sustainability 13(9):5031. https://doi.org/10.3390/su13095031
Jeske O, Jogler M, Petersen J, Sikorski J, Jogler C (2013) From genome mining to phenotypic microarrays: Planctomycetes as source for novel bioactive molecules. Antonie Van Leeuwenhoek 104(4):551–567. https://doi.org/10.1007/s10482-013-0007-1
Jeske O, Schuler M, Schumann P, Schneider A, Boedeker C, Jogler M, Bollschweiler D, Rohde M, Mayer C, Engelhardt H, Spring S, Jogler C (2015) Planctomycetes do possess a peptidoglycan cell wall. Nat Commun 6:7116. https://doi.org/10.1038/ncomms8116
Jeske O, Surup F, Ketteniß M, Rast P, Förster B, Jogler M, Wink J, Jogler C (2016) Developing techniques for the utilization of Planctomycetes as producers of bioactive molecules. Front Microbiol. https://doi.org/10.3389/fmicb.2016.01242
Jogler C, Glöckner Frank O, Kolter R (2011) Characterization of Planctomyces limnophilus and development of genetic tools for its manipulation establish it as a model species for the phylum Planctomycetes. Appl Environ Microbiol 77(16):5826–5829. https://doi.org/10.1128/AEM.05132-11
Jogler C, Waldmann J, Huang X, Jogler M, Glöckner FO, Mascher T, Kolter R (2012) Identification of proteins likely to be involved in morphogenesis, cell division, and signal transduction in Planctomycetes by comparative genomics. J Bacteriol 194(23):6419–6430. https://doi.org/10.1128/JB.01325-12
Kallscheuer N, Jogler C (2021) The bacterial phylum Planctomycetes as novel source for bioactive small molecules. Biotechnol Adv 53:107818. https://doi.org/10.1016/j.biotechadv.2021.107818
Kallscheuer N, Moreira C, Airs R, Llewellyn CA, Wiegand S, Jogler C, Lage OM (2019) Pink-and orange-pigmented Planctomycetes produce saproxanthin-type carotenoids including a rare C45 carotenoid. Environ Microbiol Rep 11(6):741–748. https://doi.org/10.1111/1758-2229.12796
Kallscheuer N, Jeske O, Sandargo B, Boedeker C, Wiegand S, Bartling P, Jogler M, Rohde M, Petersen J, Medema MH, Surup F, Jogler C (2020) The planctomycete Stieleria maiorica Mal15T employs stieleriacines to alter the species composition in marine biofilms. Commun Biol 3(1):303. https://doi.org/10.1038/s42003-020-0993-2
Kallscheuer N, Rast P, Jogler M, Wiegand S, Kohn T, Boedeker C, Jeske O, Heuer A, Quast C, Glöckner FO, Rohde M, Jogler C (2021) Analysis of bacterial communities in a municipal duck pond during a phytoplankton bloom and isolation of Anatilimnocola aggregata gen. nov., sp. nov., Lacipirellula limnantheis sp. nov. and Urbifossiella limnaea gen. nov., sp. nov. belonging to the phylum Planctomycetes. Environ Microbiol 23(3):1379–1396. https://doi.org/10.1111/1462-2920.15341
Kallscheuer N, Wurzbacher CE, Schmitz RA, Jogler C (2024) In the footsteps of Heinz Schlesner and Peter Hirsch: exploring the untapped diversity of the phylum Planctomycetota in isolates from the 1980s to the early 2000s. Syst Appl Microbiol 47(1):126486. https://doi.org/10.1016/j.syapm.2023.126486
Klimek D, Herold M, Calusinska M (2024) Comparative genomic analysis of Planctomycetota potential for polysaccharide degradation identifies biotechnologically relevant microbes. BMC Genomics 25(1):523. https://doi.org/10.1186/s12864-024-10413-z
Kohn T, Rast P, Kallscheuer N, Wiegand S, Boedeker C, Jetten MSM, Jeske O, Vollmers J, Kaster A-K, Rohde M, Jogler M, Jogler C (2020) The microbiome of Posidonia oceanica seagrass leaves can be dominated by Planctomycetes. Front Microbiol 11:1458. https://doi.org/10.3389/fmicb.2020.01458
Kremers G-J, Goedhart J, van Munster EB, Gadella TWJ (2006) Cyan and yellow super fluorescent proteins with improved brightness, protein folding, and FRET Förster radius. Biochemistry 45(21):6570–6580. https://doi.org/10.1021/bi0516273
Kruse L, Loeschcke A, de Witt J, Wierckx N, Jaeger K-E, Thies S (2024) Halopseudomonas species: cultivation and molecular genetic tools. Microbial Biotechnol 17(1):e14369. https://doi.org/10.1111/1751-7915.14369
Lachnit T, Fischer M, Kunzel S, Baines JF, Harder T (2013) Compounds associated with algal surfaces mediate epiphytic colonization of the marine macroalga Fucus vesiculosus. FEMS Microbiol Ecol 84(2):411–420. https://doi.org/10.1111/1574-6941.12071
Lage OM, Bondoso J (2014) Planctomycetes and macroalgae, a striking association. Front Microbiol 5:267. https://doi.org/10.3389/fmicb.2014.00267
Li H, Durbin R (2009) Fast and accurate short read alignment with Burrows-Wheeler transform. Bioinformatics 25(14):1754–1760. https://doi.org/10.1093/bioinformatics/btp324
Li H, Durbin R (2010) Fast and accurate long-read alignment with Burrows-Wheeler transform. Bioinformatics 26(5):589–595. https://doi.org/10.1093/bioinformatics/btp698
Mahajan M, Seeger C, Yee B, Andersson SGE (2020) Evolutionary remodeling of the cell envelope in bacteria of the Planctomycetes phylum. Genome Biol Evol 12(9):1528–1548. https://doi.org/10.1093/gbe/evaa159
Meiresonne NY, van der Ploeg R, Hink MA, den Blaauwen T (2017) Activity-related conformational changes in d, d-carboxypeptidases revealed by in vivo periplasmic Förster resonance energy transfer assay in Escherichia coli. mBio 8(5):10–1128. https://doi.org/10.1128/mbio.01089-17.10.1128/mbio.01089-17
Meiresonne NY, Consoli E, Mertens LMY, Chertkova AO, Goedhart J, den Blaauwen T (2019) Superfolder mTurquoise2ox optimized for the bacterial periplasm allows high efficiency in vivo FRET of cell division antibiotic targets. Mol Microbiol 111(4):1025–1038. https://doi.org/10.1111/mmi.14206
Meyers A, Furtmann C, Gesing K, Tozakidis IEP, Jose J (2019) Cell density-dependent auto-inducible promoters for expression of recombinant proteins in Pseudomonas putida. Microbial Biotechnol 12(5):1003–1013. https://doi.org/10.1111/1751-7915.13455
Milke L, Kabuu M, Zschoche R, Gatgens J, Krumbach K, Carlstedt KL, Wurzbacher CE, Balluff S, Beemelmanns C, Jogler C, Marienhagen J, Kallscheuer N (2024) A type III polyketide synthase cluster in the phylum Planctomycetota is involved in alkylresorcinol biosynthesis. Appl Microbiol Biotechnol 108(1):239. https://doi.org/10.1007/s00253-024-13065-x
Olson DG, Maloney M, Lanahan AA, Hon S, Hauser LJ, Lynd LR (2015) Identifying promoters for gene expression in Clostridium thermocellum. Metab Eng Commun 2:23–29. https://doi.org/10.1016/j.meteno.2015.03.002
Panter F, Garcia R, Thewes A, Zaburannyi N, Bunk B, Overmann J, Gutierrez MV, Krug D, Müller R (2019) Production of a dibrominated aromatic secondary metabolite by a Planctomycete implies complex interaction with a macroalgal host. ACS Chem Biol 14(12):2713–2719. https://doi.org/10.1021/acschembio.9b00641
Pátek M, Nešvera J, Guyonvarch A, Reyes O, Leblon G (2003) Promoters of Corynebacterium glutamicum. J Biotechnol 104(1):311–323. https://doi.org/10.1016/S0168-1656(03)00155-X
Pédelacq J-D, Cabantous S, Tran T, Terwilliger TC, Waldo GS (2006) Engineering and characterization of a superfolder green fluorescent protein. Nat Biotechnol 24(1):79–88. https://doi.org/10.1038/nbt1172
Quiñonero-Coronel MdM, Devos DP, Garcillán-Barcia MP (2024) Specificities and commonalities of the Planctomycetes plasmidome. Environ Microbiol 26(5):e16638. https://doi.org/10.1111/1462-2920.16638
Rivas-Marín E, Canosa I, Santer E, Devos DP (2016) Development of genetic tools for the manipulation of the planctomycetes. Front Microbiol 7:914
Rivas-Marin E, Peeters SH, Claret Fernandez L, Jogler C, van Niftrik L, Wiegand S, Devos DP (2020) Non-essentiality of canonical cell division genes in the planctomycete Planctopirus limnophila. Sci Rep 10(1):66. https://doi.org/10.1038/s41598-019-56978-8
Rivas-Marin E, Moyano-Palazuelo D, Henriques V, Merino E, Devos DP (2023) Essential gene complement of Planctopirus limnophila from the bacterial phylum Planctomycetes. Nat Commun 14(1):7224. https://doi.org/10.1038/s41467-023-43096-3
Robinson JT, Thorvaldsdóttir H, Wenger AM, Zehir A, Mesirov JP (2017) Variant review with the integrative genomics viewer. Cancer Res 77(21):e31–e34. https://doi.org/10.1158/0008-5472.CAN-17-0337
RStudio (2022) RStudio Team, RStudio: integrated development environment for R. RStudio, PBC, Boston, MA, URL http://www.rstudio.com/
Sambrook J, Russell D (2001) Molecular cloning, vol. 1–3. Cold Spring Harbor Laboratory Press, Cold Spring Harbor, NY
Sandargo B, Jeske O, Boedeker C, Wiegand S, Wennrich J-P, Kallscheuer N, Jogler M, Rohde M, Jogler C, Surup F (2020) Stieleriacines N-acyl dehydrotyrosines from the marine Planctomycete Stieleria neptunia sp. nov. Front Microbiol 11:1408. https://doi.org/10.3389/fmicb.2020.01408
Santana-Molina C, Henriques V, Hornero-Mendez D, Devos DP, Rivas-Marin E (2022) The squalene route to C30 carotenoid biosynthesis and the origins of carotenoid biosynthetic pathways. Proc Natl Acad Sci USA 119(52):e2210081119. https://doi.org/10.1073/pnas.2210081119
Schindelin J, Arganda-Carreras I, Frise E, Kaynig V, Longair M, Pietzsch T, Preibisch S, Rueden C, Saalfeld S, Schmid B, Tinevez J-Y, White DJ, Hartenstein V, Eliceiri K, Tomancak P, Cardona A (2012) Fiji: an open-source platform for biological-image analysis. Nat Methods 9(7):676–682. https://doi.org/10.1038/nmeth.2019
Seemann T (2014) Prokka: rapid prokaryotic genome annotation. Bioinformatics 30(14):2068–2069. https://doi.org/10.1093/bioinformatics/btu153
Shaner NC, Campbell RE, Steinbach PA, Giepmans BNG, Palmer AE, Tsien RY (2004) Improved monomeric red, orange and yellow fluorescent proteins derived from Discosoma sp. red fluorescent protein. Nat Biotechnol 22(12):1567–1572. https://doi.org/10.1038/nbt1037
Shaner NC, Lambert GG, Chammas A, Ni Y, Cranfill PJ, Baird MA, Sell BR, Allen JR, Day RN, Israelsson M, Davidson MW, Wang J (2013) A bright monomeric green fluorescent protein derived from Branchiostoma lanceolatum. Nat Methods 10(5):407–409. https://doi.org/10.1038/nmeth.2413
Skerra A (1994) Use of the tetracycline promoter for the tightly regulated production of a murine antibody fragment in Escherichia coli. Gene 151:131–135. https://doi.org/10.1016/0378-1119(94)90643-2
Stansen C, Uy D, Delaunay S, Eggeling L, Goergen JL, Wendisch VF (2005) Characterization of a Corynebacterium glutamicum lactate utilization operon induced during temperature-triggered glutamate production. Appl Environ Microbiol 71:5920–5928. https://doi.org/10.1128/aem.71.10.5920-5928.2005
The Galaxy Community (2022) The Galaxy platform for accessible, reproducible and collaborative biomedical analyses: 2022 update. Nucleic Acids Res 50(W1):W345–W351. https://doi.org/10.1093/nar/gkac247
Van Teeseling MC, Mesman RJ, Kuru E, Espaillat A, Cava F, Brun YV, VanNieuwenhze MS, Kartal B, Van Niftrik L (2015) Anammox Planctomycetes have a peptidoglycan cell wall. Nat Commun 6:6878. https://doi.org/10.1038/ncomms7878
Vollmers J, Frentrup M, Rast P, Jogler C, Kaster AK (2017) Untangling genomes of novel planctomycetal and verrucomicrobial species from Monterey Bay kelp forest metagenomes by refined binning. Front Microbiol 8:472. https://doi.org/10.3389/fmicb.2017.00472
Walker BJ, Abeel T, Shea T, Priest M, Abouelliel A, Sakthikumar S, Cuomo CA, Zeng Q, Wortman J, Young SK, Earl AM (2014) Pilon: an integrated tool for comprehensive microbial variant detection and genome assembly improvement. PLoS ONE 9(11):e112963. https://doi.org/10.1371/journal.pone.0112963
Wickham H (2016) ggplot2: elegant graphics for data analysis. Springer-Verlag New York. https://ggplot2.tidyverse.org. Accessed 10 June 2024
Wiegand S, Jogler M, Jogler C (2018) On the maverick Planctomycetes. FEMS Microbiol Rev 42(6):739–760. https://doi.org/10.1093/femsre/fuy029
Wiegand S, Jogler M, Boedeker C, Pinto D, Vollmers J, Rivas-Marín E, Kohn T, Peeters SH, Heuer A, Rast P, Oberbeckmann S, Bunk B, Jeske O, Meyerdierks A, Storesund JE, Kallscheuer N, Lücker S, Lage OM, Pohl T, Merkel BJ, Hornburger P, Müller R-W, Brümmer F, Labrenz M, Spormann AM, Op den Camp HJM, Overmann J, Amann R, Jetten MSM, Mascher T, Medema MH, Devos DP, Kaster A-K, Øvreås L, Rohde M, Galperin MY, Jogler C (2020) Cultivation and functional characterization of 79 planctomycetes uncovers their unique biology. Nat Microbiol 5(1):126–140. https://doi.org/10.1038/s41564-019-0588-1
Wurzbacher CE, Haufschild T, Hammer J, van Teeseling MCF, Kallscheuer N, Jogler C (2024) Planctoellipticum variicoloris gen. nov., sp. nov., a novel member of the family Planctomycetaceae isolated from wastewater of the aeration lagoon of a sugar processing plant in Northern Germany. Sci Rep 14(1):5741. https://doi.org/10.1038/s41598-024-56373-y
Zacharias DA, Violin JD, Newton AC, Tsien RY (2002) Partitioning of lipid-modified monomeric GFPs into membrane microdomains of live cells. Science 296(5569):913–916. https://doi.org/10.1126/science.1068539
Acknowledgements
The authors would like to thank Dr. Thomas Drepper (Institute for Molecular Enzyme Technology (IMET), Forschungszentrum Jülich GmbH, Germany) for providing the plasmids harboring the salicylic-acid inducible gene expression system, the group of Prof. Dr. Kai Papenfort (Microbial Gene Regulation, Friedrich Schiller University Jena) and Dr. Kathrin Fröhlich (Bacterial RNA Biology, Friedrich Schiller University Jena) for the gift of the plasmid harboring sfgfp, and Dr. Muriel van Teeseling for the gift of plasmids harboring mVenus, mCherry, and mNeonGreen. Plasmid sfTq2ox-N1 was a gift from Tanneke den Blaauwen (Addgene plasmid #117930).
Funding
Open Access funding enabled and organized by Projekt DEAL. This work was funded by the Deutsche Forschungsgemeinschaft (DFG, German Research Foundation) under Project-ID 239748522–CRC 1127 ChemBioSys (project A07), by the Deutsche Forschungsgemeinschaft under Germany’s Excellence Strategy–EXC 2051–Project-ID 390713860, by the Landesgraduiertenstipendium of the Free State of Thuringia awarded by the Friedrich Schiller University Jena, and by the Carl Zeiss Stiftung. JH and TH acknowledge funding and support by the Jena School for Microbial Communication.
Author information
Authors and Affiliations
Contributions
TH and NK performed the project planning and in silico cloning; TH, NK, NR, and VP constructed the plasmids and strains; TH performed microscopy, image analysis, and plate reader analyses; JH performed genomic analyses; TH and NK wrote the manuscript; CJ contributed to text preparation and supervised the study together with NK. All authors discussed the results, contributed to the final manuscript, and agreed with the submission.
Corresponding authors
Ethics declarations
Ethical approval
This article does not contain any studies with human participants or animals performed by any of the authors.
Conflict of interest
The authors declare no competing interests.
Additional information
Publisher's Note
Springer Nature remains neutral with regard to jurisdictional claims in published maps and institutional affiliations.
Supplementary Information
Below is the link to the electronic supplementary material.
Rights and permissions
Open Access This article is licensed under a Creative Commons Attribution 4.0 International License, which permits use, sharing, adaptation, distribution and reproduction in any medium or format, as long as you give appropriate credit to the original author(s) and the source, provide a link to the Creative Commons licence, and indicate if changes were made. The images or other third party material in this article are included in the article's Creative Commons licence, unless indicated otherwise in a credit line to the material. If material is not included in the article's Creative Commons licence and your intended use is not permitted by statutory regulation or exceeds the permitted use, you will need to obtain permission directly from the copyright holder. To view a copy of this licence, visit http://creativecommons.org/licenses/by/4.0/.
About this article
Cite this article
Haufschild, T., Hammer, J., Rabold, N. et al. Novel tools for genomic modification and heterologous gene expression in the phylum Planctomycetota. Appl Microbiol Biotechnol 109, 79 (2025). https://doi.org/10.1007/s00253-025-13462-w
Received:
Revised:
Accepted:
Published:
DOI: https://doi.org/10.1007/s00253-025-13462-w